#pathtozerocarbon, #sustainability
06 – Buildings, Energy Use + Carbon
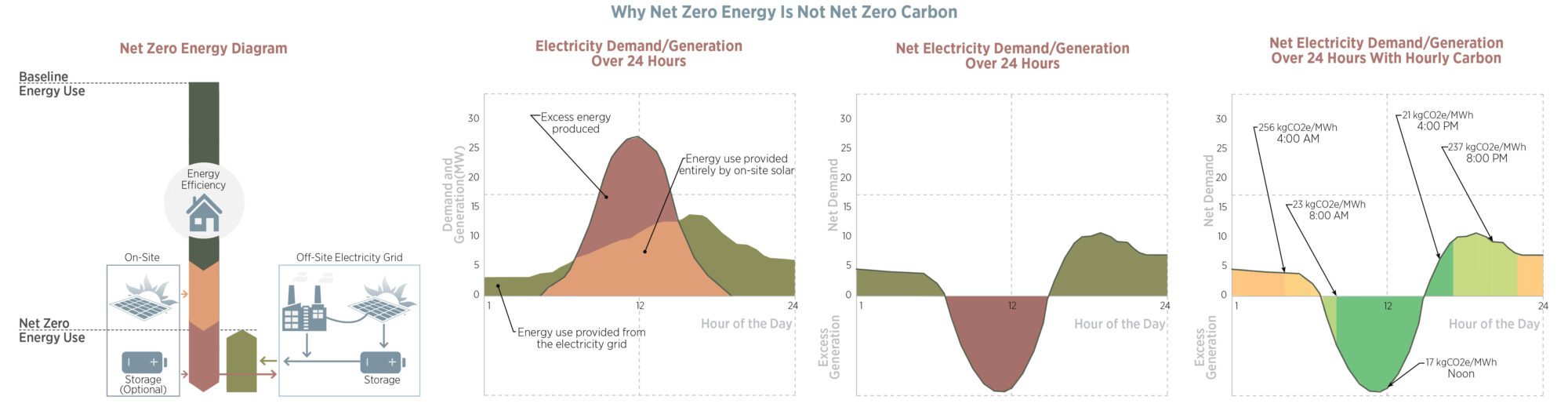
While most people think Net Zero is simply adding lots of PV panels to a building, it’s much more complicated. Nearly all buildings are connected to the electricity grid, which has a complex carbon emissions story, with electricity being generated, stored, and traded laterally, the subject of this post. We must include emissions from all these lateral trades. Based on diagrams by the New Buildings Institute.
Net Zero Energy Use ≠ Net Zero Emissions
The Design and Construction industry has rightly focused on energy use reductions for decades: our clients pay directly for energy consumption, and it has been a good proxy for operational carbon emissions. However, with goals of carbon neutrality we now need to also understand and demand that the energy supply also meets our clients’ needs. Since roughly three-quarters of electricity is used at buildings in the US (and more is likely as EVs become commonplace) we need to have a commensurate voice in the supply of low-carbon energy sources.
It is nearly impossible to consume only renewable energy at all hours of the year, a basic requirement for carbon neutrality. While many corporations are buying 100% renewable power on an annual basis, a handful of companies (such as Google) are trying to match the time of day that renewable energy is generated (and purchased) to the time energy is used in each market, currently a very challenging goal.
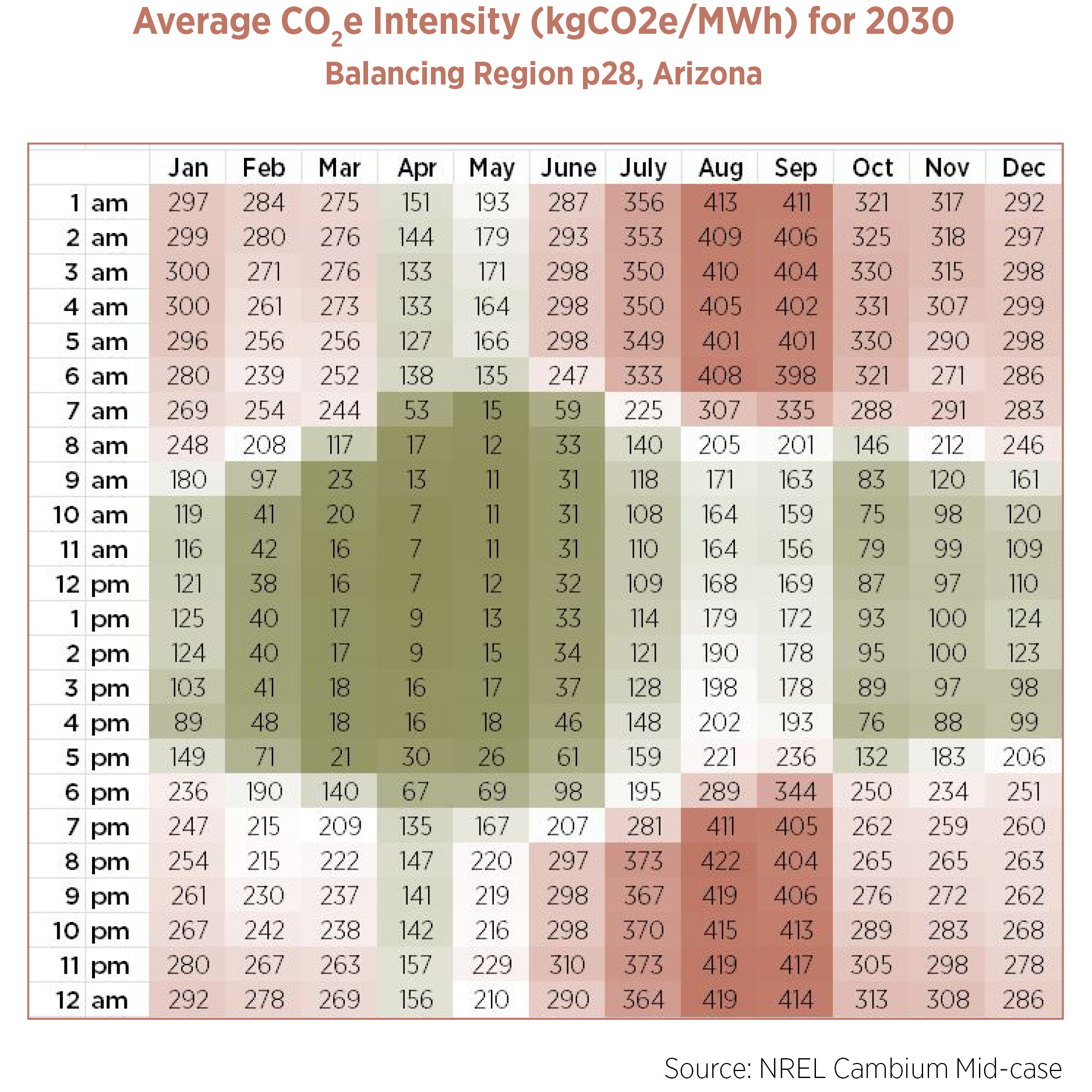
Estimated average carbon intensity of the electricity load for central Arizona in 2030 by hour and month, from NREL Cambium (mid case scenario). This shows very low values in the spring when solar is plentiful and cooling loads are low, with higher carbon intensity year-round at night. Peak carbon intensity occurs during hot summer nights when no solar resources are available and nighttime outdoor temperatures are in the upper 80s and 90s °F.
Several things have changed in the last decade that mean we need to understand both energy and carbon emissions:
- Many building owners are demanding carbon emissions reductions that we can deliver if we understand carbon and buildings.
- Energy codes, technology and design have driven down building energy use significantly per square foot area. AIA 2030 Commitment Reporting shows a change in average energy use reduction from 35% to 51% over the years 2010 to 2020, across 3.5 billion square feet.
- Electricity generation is getting cleaner in the US and worldwide.
- New electricity generation is mostly renewable energy that relies on solar and wind and is not available sometimes. This means that carbon emissions from electricity vary hourly. A low-carbon building not only uses less energy, it draws energy at the right times of each day when renewable energy is being produced.
- Reduced electricity use due to efficiency decreases the cost of the renewable energy transition and frees up electricity generation capacity for electrifying transportation.
- Many low-energy systems leak refrigerants with high global warming potential, partially offsetting their advantage, so we need to translate this into carbon equivalent emissions to make decisions.
Electricity v On-Site Fossil Fuel Combustion
While most of the electricity in the US still comes from fossil fuels, this is quickly trending towards renewables, with many states in the US, accounting for more than half of the country’s electricity, committed to use 100% renewable energy by 2050. While the average carbon intensity of electricity will decrease, on-site combustion of fossil fuels like natural gas are not expected to reduce their carbon intensity significantly, and are likely underreporting emissions since they typically do not account for the potent methane leaks at wellheads and from gas pipelines. Renewably harvested gas is likely to be prioritized for transportation as it is lightweight and can fuel freight vehicles more easily than current batteries. While the hydrogen economy has been hypothesized for decades, unfortunately most gas pipelines and end use equipment are not able to handle hydrogen without significant modification and cost. Hydrogen will play a role in a carbon-free economy, but the highest priority for green hydrogen is not likely at the building scale. Put simply, efficient, all-electric buildings will trend toward zero carbon while buildings that consume fossil fuels on site will not.
This post is primarily about electricity and carbon emissions for two reasons: carbon emissions from fossil fuels are fairly straight-forward to calculate, and efficient electrification is a critical part of the long-term path towards a carbon neutral built environment.
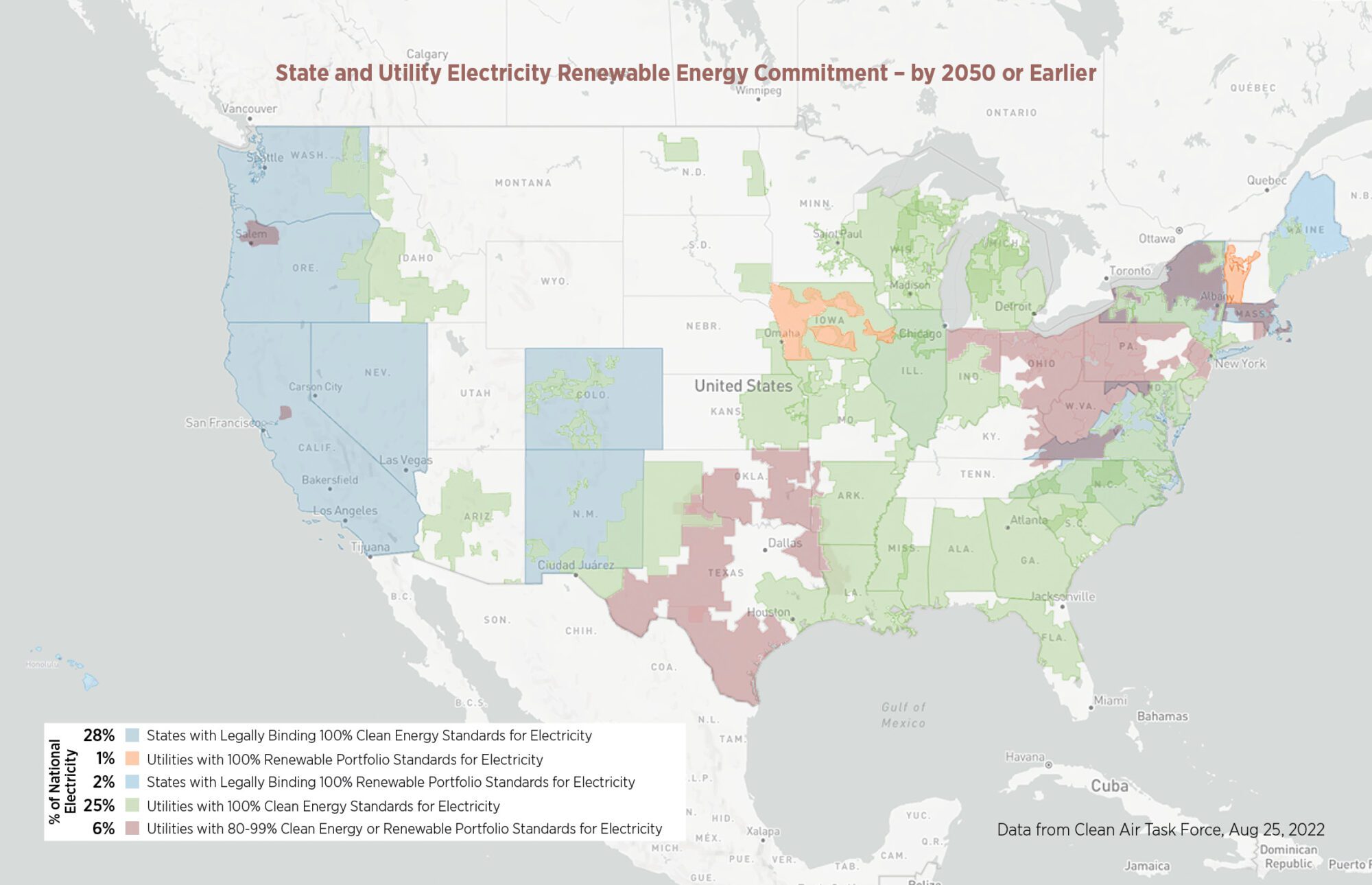
Over 50% of US energy supply is required or committed to be 100% renewable before 2050, with a further 10% of the grid committed to 80% renewable by then. Renewable energy is now the lowest-cost new energy source in much of the US.
Where does my Electricity come from?
An increasing number of buildings owners are buying off-site renewable energy, often through contracts like Virtual Power Purchase Agreements (VPPAs). Since renewable energy, once built, has no direct fuel costs, a long-term arrangement for 100% renewable energy can be cheaper than buying energy from the utility. The location and type of power being generated through these are important to the carbon emissions from a project, as we see in the next few sections.
While we sometimes talk about the US electricity grid carbon emissions, the grid is not managed on a national scale. Instead, there are roughly 66 balancing authorities, and many of these are managed by larger entities called Regional Transmission Organizations (RTOs) or Independent System Operators (ISOs). These entities decide when to turn power plants on, off, up or down, and buy or sell power entities at each minute of each day in a complex dance. For example, when electricity demand is high for the Public Service Company of New Mexico Balancing Authority, they can generate more power or buy it from Arizona Public Service Company or many other nearby Balancing Authorities. Electricity is also generated from privately owned rooftop solar; one analysis shows the lowest cost electricity grid has a substantial amount of rooftop solar.
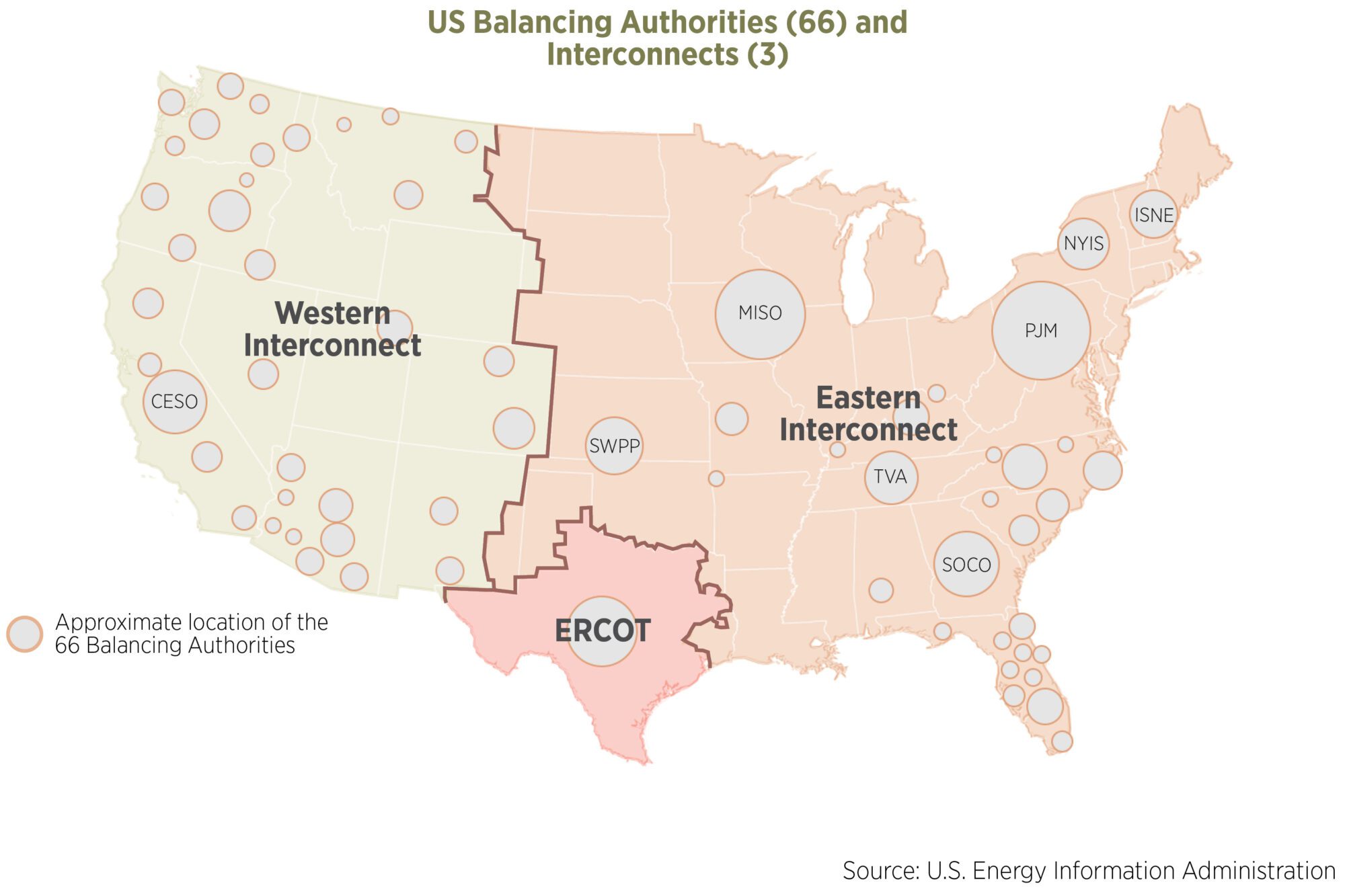
The US electricity sector has many scales: There are 3 largely independent ‘Interconnects,’ made up of 66 balancing authorities that manage power regionally. The balancing authorities determine which power plants generate electricity and trade with one another to maintain the right amount of electricity supply to meet demand.
At a larger scale, there are three major grid regions in the continental US and lower Canada: the Eastern, Western, and ERCOT (Texas) Interconnections. They are largely independent, and only very limited amounts of power can be transferred between the three regions. The Western Interconnect also includes an Energy Imbalance Market as a real-time power market to reduce cost and carbon that spans many balancing authorities.
New generation often requires upgrades to electricity transmission lines since renewable energy is location-dependent, and this is one of the larger problems in deploying new renewable energy: over 2,000 GigaWatts of planned new generation (95% renewable) is awaiting transmission connection approval.
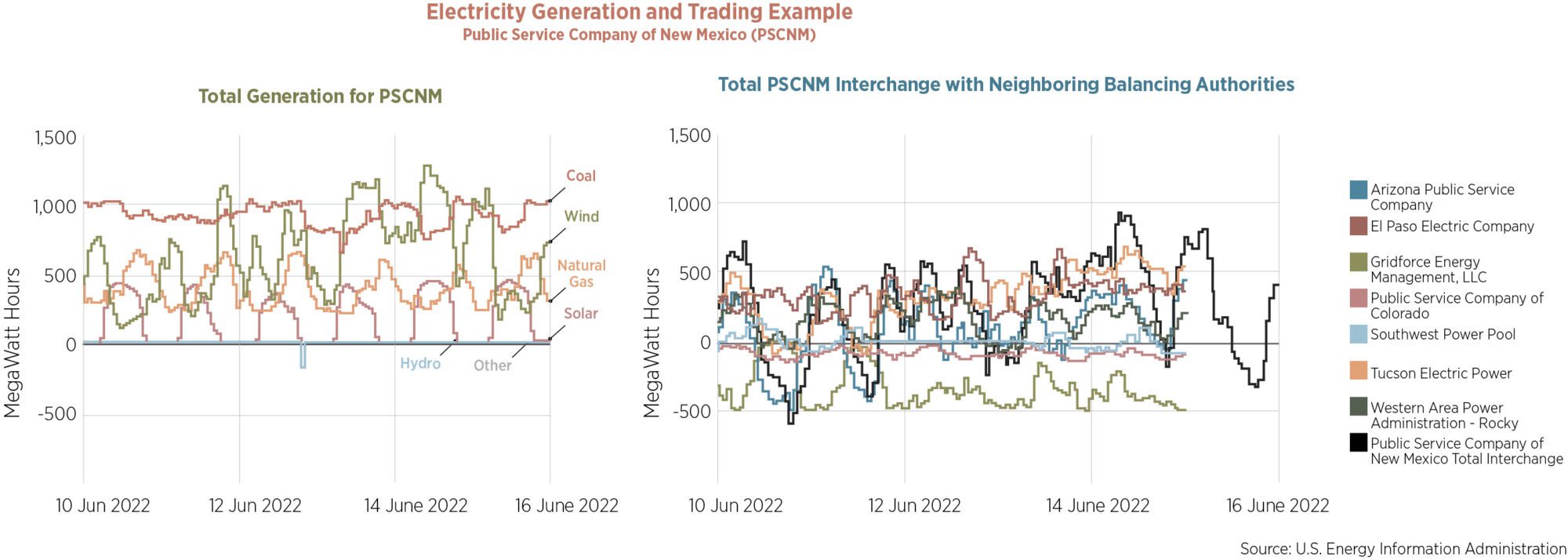
One balancing region – the Public Service Company of New Mexico – shows the varying generation over 6 days (left graph), with solar, wind and gas generation varying significantly throughout each day. The right graph shows the complexity of electricity being traded with other nearby balancing authorities throughout each day to maintain the right electricity supply. Data from these two graphs informs actual hourly carbon profiles.
How the Grid Generates Electricity: Baseload, Intermittent, and Dispatchable
Balancing authorities often use cost determining which power plants to use. Data Analytics, including weather forecasts help them project the minute-by-minute energy use days ahead of time so they can match demand with supply.
- Baseload generation typically runs full capacity 24 hours a day. They are relatively inexpensive to operate but can take around a day (coal) or a week (nuclear) to turn on and off. As intermittent renewables become more common, baseload generation is becoming less prevalent. Common types: Nuclear, Coal.
- Intermittent generation runs whenever weather conditions are powering renewables (primarily solar and wind), but the power generation cannot be increased by grid operators. This type is very cheap to operate since there is no fuel input, so it is rarely turned off (called curtailment). Intermittent generation requires electricity storage, dispatchable generation, or a transmission network that supplies a large geographic region to maintain grid consistency. Common types: Solar, Wind.
- Storage can be used to limit the need for high-carbon dispatchable generation and can be at the utility-scale or at the building scale. Common Types: Pumped Hydro. Utility-scale batteries and hydrogen are emerging technologies. Electric vehicles can also provide electricity storage.
- Dispatchable generation meets the remainder of the electricity load not supplied by storage, baseload or intermittent generation. These power plants are ramped up and down to meet the hour-by-hour and, in some cases, the minute-by-minute load changes induced by the grid. Load-following power plants are turned on and off on a daily basis, while peaking power (peaker) plants are only used at the very highest (peak) electricity use, perhaps only for a few hours during a few days of the year, and are the least efficient and highest emissions sources. Common Types: Natural Gas, Diesel (Hawaii and Alaska), and Hydro. Geothermal can also be used this way.
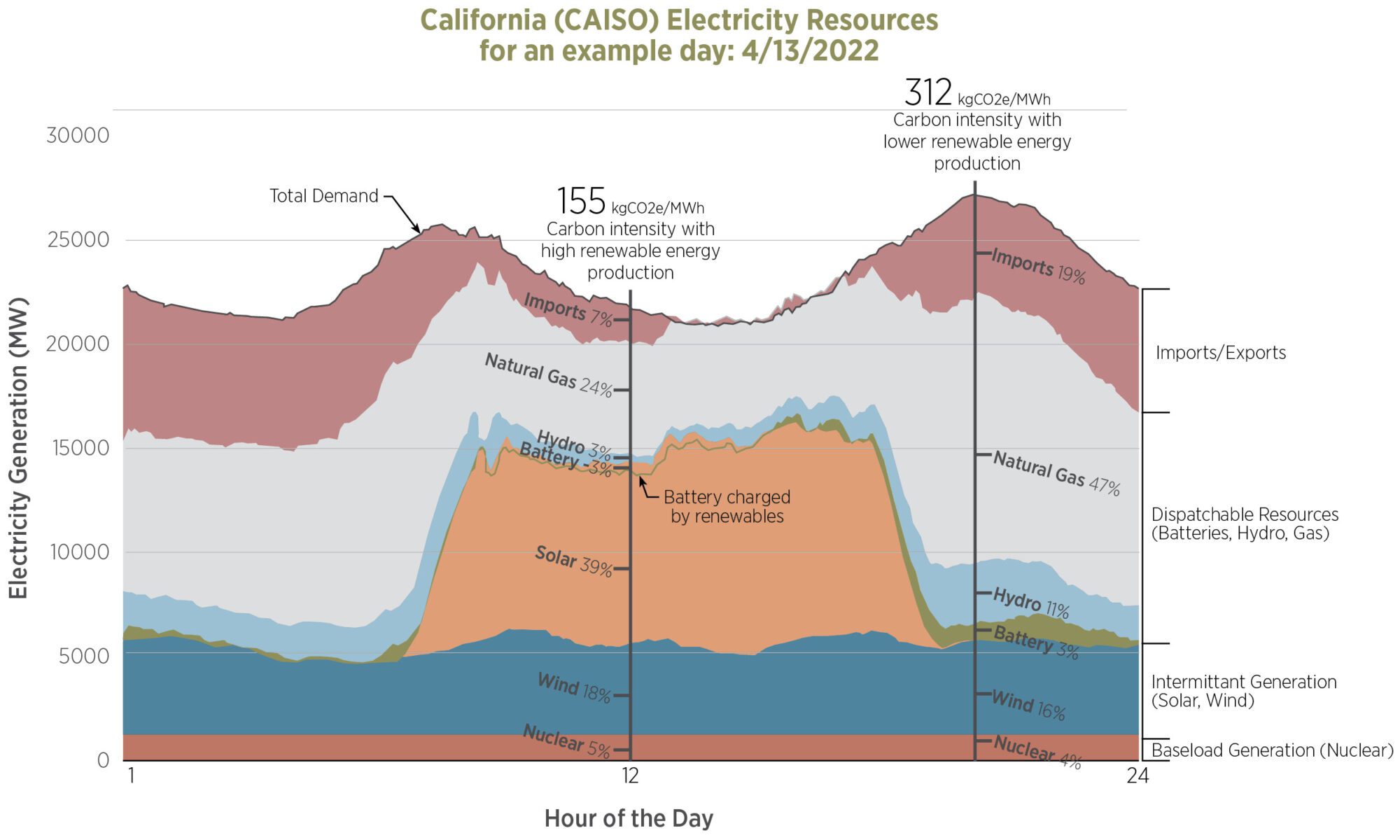
The California (CAISO) electricity grid is indicative of solar and wind-reliant grids. Constant baseload generation is challenging in a region with abundant renewable energy, leading to steep ramping of dispatchable resources when the sun goes down. As more renewables are added, energy storage, energy efficiency, and grid-interactive buildings will help manage energy transfer, reducing the necessity for dispatchable fossil fuel generation. Actual data for April 13, 2022 from CAISO.
Grid-Friendly Buildings
To reduce the emissions from dispatchable generation and the embodied carbon associated with utility scale energy storage, buildings can be made to work with the new, renewable electricity grid. This will have a huge impact on the cost of the renewable energy transition since buildings use three-quarters of US electricity.
Grid-Interactive buildings can help in two ways:
- Consume electricity when renewables resources are generating. This is the most effective grid-interactive carbon reduction strategy, as it occurs every day. Architects should review the ‘load shapes’ (diagram below) for typical days to see where grid-interactive strategies can beneficially shift electricity demand.
- Move loads away from times when an entire region is at peak demand. These occur perhaps 80 hours a year (often hot summer afternoons and evenings as well as cold winter mornings) but require the construction of peaker electricity plants or electricity storage, which both have high embodied carbon footprints.
The simplest strategies to make buildings grid-interactive, reducing carbon emissions:
- Fenestration optimization and shading to lower peak cooling and avoid times when the electricity grid has high carbon emissions. Minimizing west-facing windows or protecting them from solar gains during these times reduces building energy use, electricity demand, and sometimes mechanical equipment size and cost. The peak load shading may be very different than the shading that provides the best annual energy use reductions.
- Lighting reductions. During peak cooling times, lowering lighting by 10% (often not noticeable) can reduce building cooling loads and overall electricity demand.
- Energy storage for water heaters. With large storage, hot water can be generated at times of day when the emissions impact of using electricity is lowest.
- Chilled water storage. Chilled water for space cooling or refrigeration can be generated at times of day when electricity carbon is lowest.
- Smart EV charging. If electric vehicles (EVs) have charging flexibility, charging can be scheduled for times when excess electricity is being generated on site, grid emission are lowest, or peak grid/draw is not occurring. Though not yet common in practice, EVs have the potential to also provide power to the building to further help control the load shape, perhaps by purchasing the energy from the EV owner.
- On-site energy generation and storage.
- Co-Generation shifts peaking generation from the utility to the customer. Most co-generation plants use fossil fuels, so while this is often a cost win at the right scale, it will not trend towards carbon neutrality.
- Appliances that are programmed to operate when lots of renewable energy is being generated.
Operating cost are also driving grid-interactivity: building owners pay for electricity use and they often pay higher rates during times of peak electricity usage (peak rates); they also increasingly pay demand charges in some areas. Demand charges are based on a building’s peak electricity demand during the previous billing period – this fee helps the utility pay the cost to build and operate peaking generation plants that are infrequently used. Reducing the peak loads (and thus demand charges) aids utilities in building out the lowest cost electricity grid possible, with less storage and fewer peaker plants. The cost of building out the renewable energy electricity grid (including storage) – and thus the cost of electricity – will depend on how grid-interactive our buildings are.
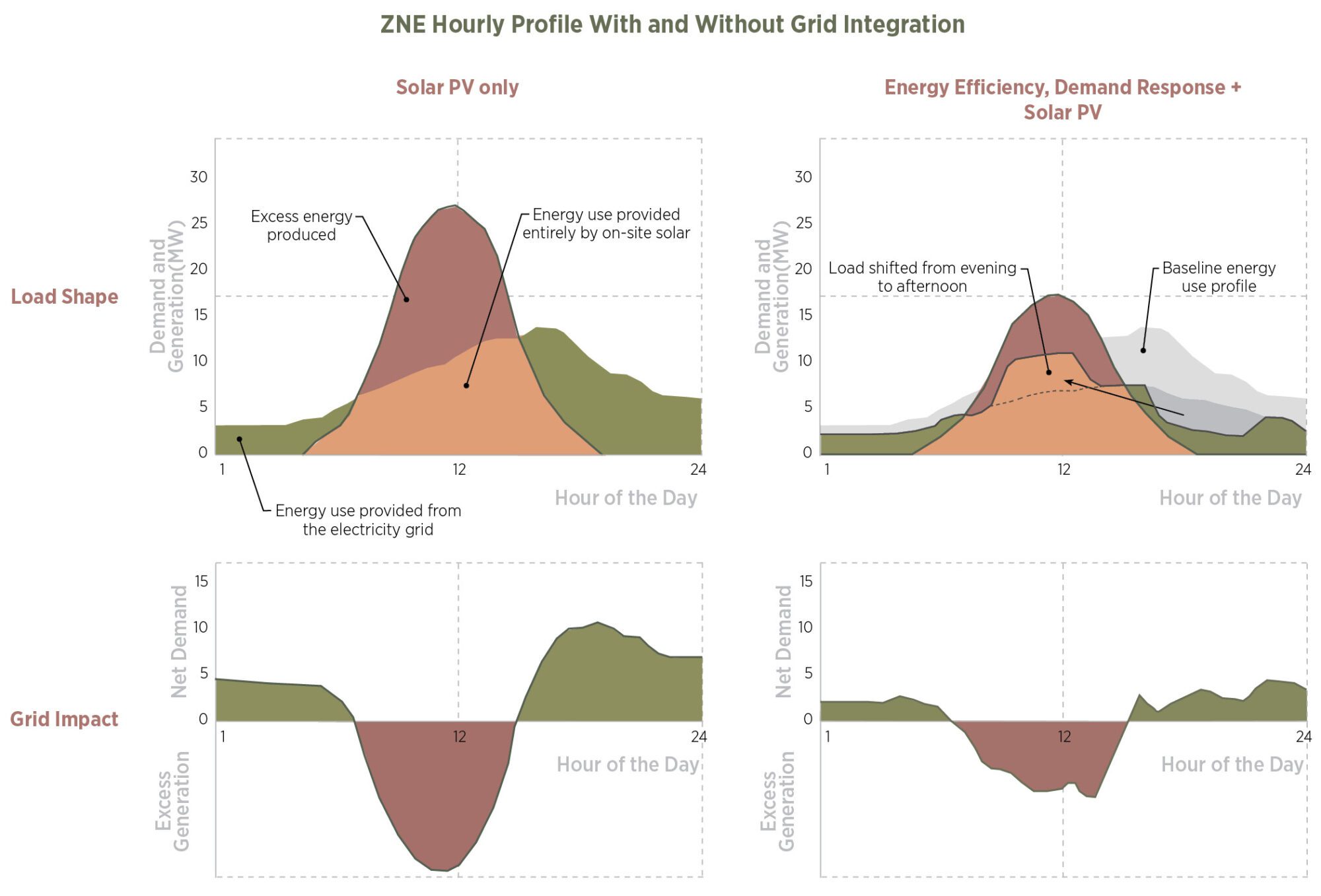
The upper left diagram shows a building’s electricity demand (load profile) and solar PV output over 24 hours; the lower left shows how the electricity grid needs to respond, providing power sometimes and then absorbing power during peak solar generation times. This demand profile is difficult and expensive for utilities to meet. On the right is a building load profile modified by energy efficiency and demand response (or load shifting). The bottom right shows a resulting load profile that is much easier and less expensive for the utility to meet since it involves less extreme peak demand and peak supply. Based on a diagram by the New Buildings Institute.
Estimating Energy + Carbon Emissions
With an understanding of how the electricity grid generates electricity, we can now talk about how to calculate its carbon emissions. There are at least three ways to measure the carbon intensity of electricity (kgCO2e/MWh):
- Average emissions include the average carbon intensity of all power produced or consumed in a region.
- Short-run marginal emissions are an estimate of the carbon intensity of the electricity grid due to immediate changes in demand, often requiring turning up or down the dirtier dispatchable generation.
- Long-run marginal emissions are an estimate of the carbon intensity of the grid due to an increase or decrease in load, considering how the change may influence the operation and structure of the grid. This could be based on a policy or code that requires efficiency or grid-interactivity from a class of buildings. Since building-sector choices tend to be long-lived, long-run marginal emissions more comprehensively describe the impact of building-scale choices.
Historically most architects have used average annual emissions during design, such as regional e-grid emissions. We now know that carbon intensity varies significantly on an hourly basis, and estimates using average annual emissions can be off by 35% or more versus using hourly emissions. Worse, annual estimates don’t reward building-scale, grid-interactive strategies that can significantly reduce energy related carbon emissions, the cost to build out the new grid, and the embodied carbon from building out the new grid.
Since buildings operate for many decades, design teams should use forward-looking emissions that include recent and planned renewable energy and other grid changes. One example, NREL’s Cambium, contains forward-looking emissions based on the complex interaction of economics, state and federal laws, regional power trading, operational requirements of the grid, and more. Tips and tricks for using Cambium to forecast emissions on projects will be part of a future post.
New and existing buildings can further reduce operational carbon through real-time grid-interactivity: WattTime provides 72 hour forecasts for the carbon intensity of local electricity so customers can time their energy use to lower carbon emissions. This means that internet-connected smart devices and building systems can automatically sync their energy usage with cleaner moments in the day. ElectricityMaps is also in this space.
From a carbon perspective, it is difficult to separate carbon emissions from grid electricity at a scale smaller than the region of the balancing authority, so our recommendation is to use this scale. We also recommend using forward-looking, average hourly emissions for carbon estimates and forward-looking, hourly long-run marginal emissions for comparing two design options.
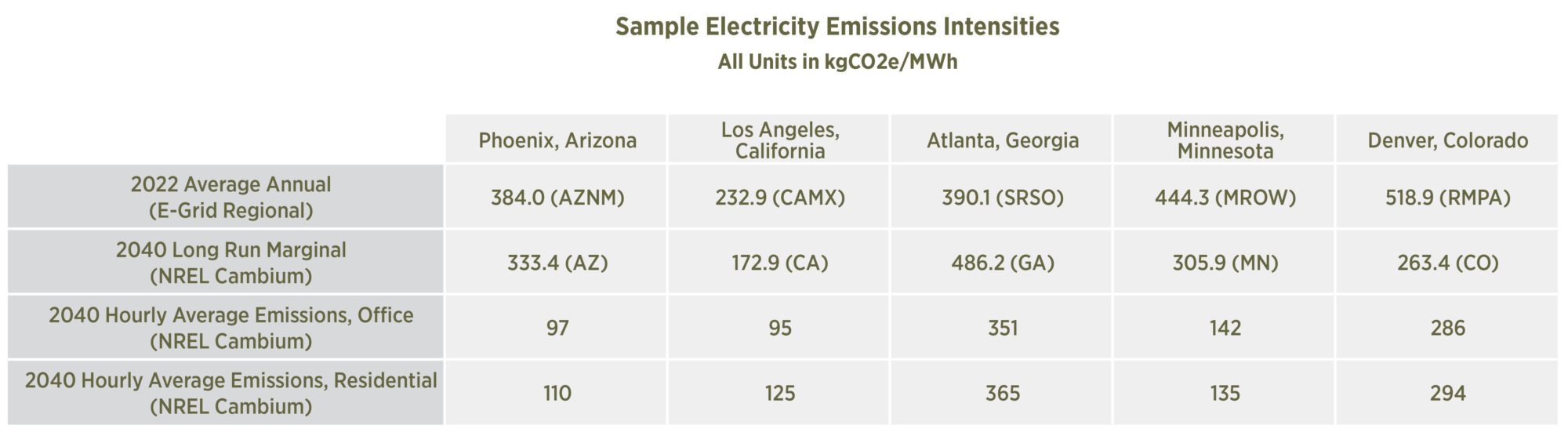
E-grid emissions are backwards looking, coming from historical power generation, used in LEED and other programs. Long Run Marginal Emissions are best used to compare two design options for carbon emissions improvements at the building scale. Since the grid is rapidly adopted renewables, LMN recommends using average annual emissions using projections like NREL Cambium. If using a single year, we recommend using the midpoint for the first 30 years of operation (2040 in this case) when initial equipment type is still likely to be used. ASHRAE prototype buildings were used for simulation, post-processed with NREL Cambium Mid-Case hourly projections.
Other Energy-related Emissions
A comprehensive accounting of a building’s carbon emissions should also include:
- Embodied carbon accounting for new renewable energy infrastructure to supply the building’s needs, even if renewable energy is not built on site. While a rough estimate, some evidence suggests crystalline PV panels have an embodied carbon in the range of 15-45 kg CO2e/MWh. For a building with 30 EUI and embodied carbon of 50 kgCO2e/ft2, the embodied carbon of new PV panels to reach net zero energy adds around 10-20% to the embodied carbon total. Off-site renewable energy has land use implications, since building out the renewable grid will take millions of acres of land, but reduce land use needs for oil and gas production.
- Pre-combustion emissions from fossil fuels and construction of power plants. Some emissions factors do not include emissions associated with methane leaks in at wellheads and from pipelines, or the emissions from extraction, refinement, and transportation of fuels. This study looks at lifetime energy loss to these factors.
- Energy systems also use significant water resources and water systems use significant energy resources, sometimes referred to as the Water-Energy Nexus. Around 40% of water use in the US is used for power plants, and cooling towers in buildings also use a great deal of water. Water collection and treatment requires significant energy use as well. A thoughtful carbon analysis should include the emissions and ecological impact of attributional and consequential water use.
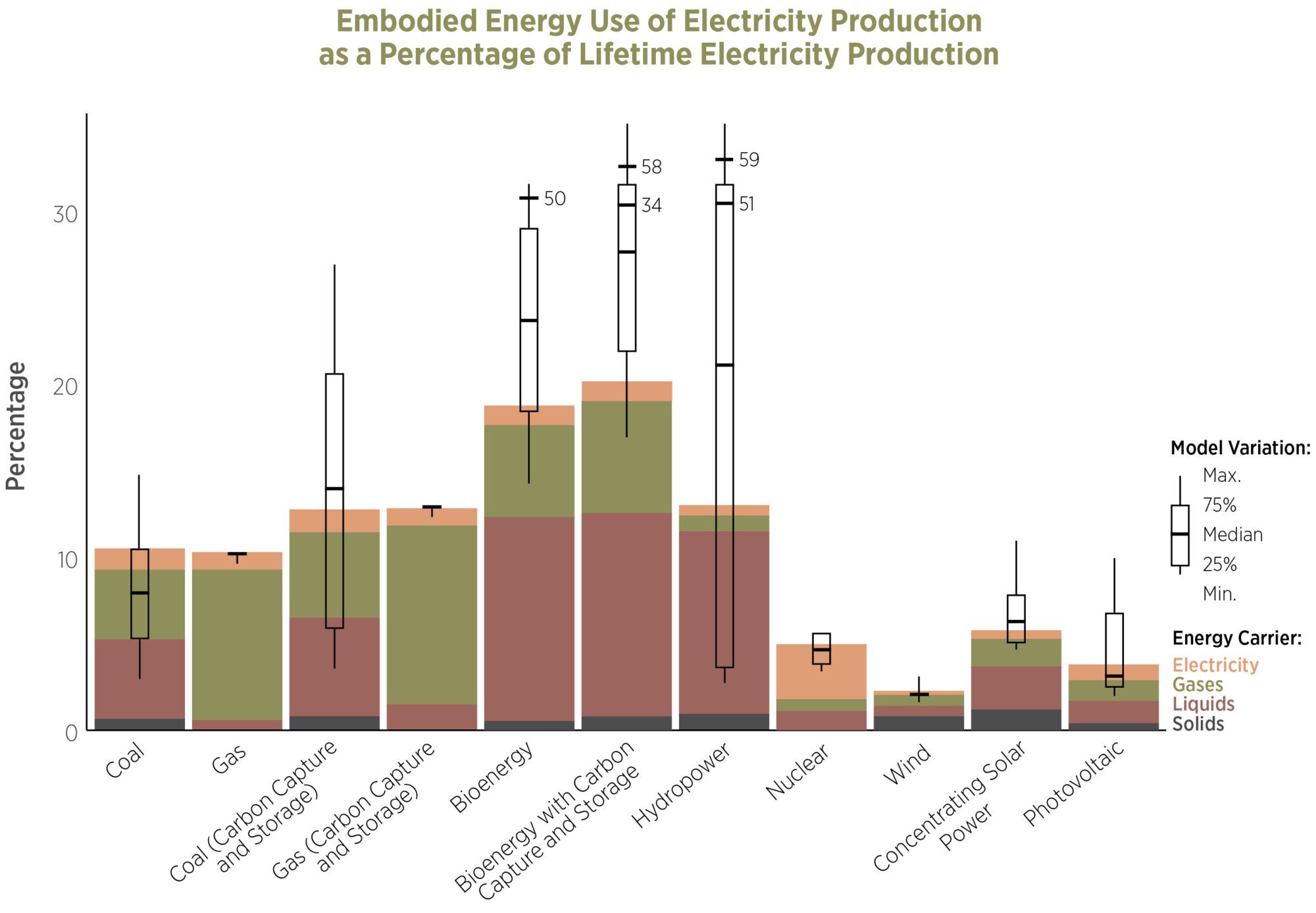
Carbon emission from electricity generation typically ignore many factors, including the embodied carbon for the construction of the power plants and production and transportation of the fuels and other inputs. A study (Pehl et all, 2017) illustrates the embodied energy for these factors as a percentage of lifetime electricity production.
Conclusions and Recommendations
Going forward, our buildings will need to be grid-interactive to reduce the carbon intensity and lower the cost of the renewable energy transition. Design teams will need to make decisions based on hourly carbon calculations, including carbon-intensity electricity forecasts for 2030 or 2050, and use balancing authority-scale data, if possible, that includes emissions from electricity demand (not just balancing authority power generation).
Future posts will detail how to do this, including NREL’s Cambium viewer for forward-looking electricity data as well the LEED ACP excel file created by NBI for GridOptimal that estimates carbon emissions reductions due to grid-interactivity.
- Use forward-looking, hourly carbon emissions profiles for electricity production and consumption at the balancing authority (or state) Co-generation requires custom electricity factors as it produces both heat and electricity
- Consider strategies that use renewable energy when it is being generated and limit energy use when it the grid has fewer renewables
- Account for the embodied carbon of new renewable energy to match consumption, even if not provided on site. Energy efficiency provides a large embodied carbon reduction, reduces land use for renewable energy, and is the primary operational carbon reduction strategy.
Please email any questions or comments to Kjell Anderson, kanderson@lmnarchitects.com
Thanks to our external collaborators and peer reviewers
Pieter Gagnon, NREL; Mark Frankel, Ecotope; Henry Richardson, WattTime; Jesse Walton, Mahlum Architects; Charles Eley, Architecture 2030; Caroline Traube, McKinstry; Dan Jaffe, University of Washington
LMN Architects Team
Huma Timurbanga, Justin Schwartzhoff, Jenn Chen, Chris Savage, Andrew Gustin, Kjell Anderson
Posted: 10/20/2022
Edited: 09/25/2023
The text, images and graphics published here should be credited to LMN Architects unless stated otherwise. Permission to distribute, remix, adapt, and build upon the material in any medium or format for noncommercial purposes is granted as long as attribution is given to LMN Architects.