#pathtozerocarbon, #sustainability
04 – The Science of Global Warming
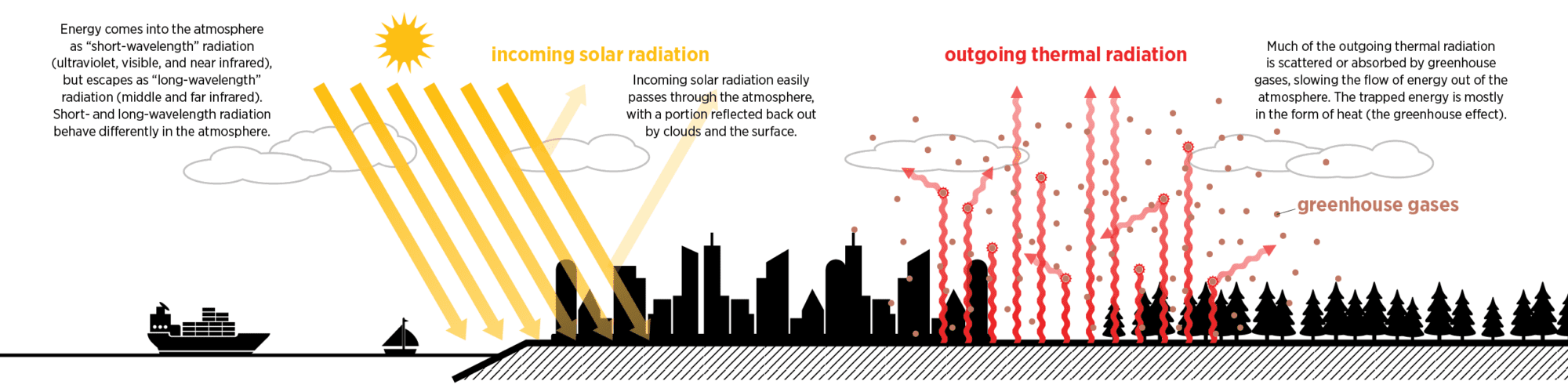
Incoming solar radiation mostly passes through the atmosphere, but outgoing thermal radiation is scattered and absorbed/reemitted, leading to a slow diffusion of the energy back out of the atmosphere: the trapped energy keeps the atmosphere warm (the greenhouse effect).
How do carbon emissions impact the earth and its inhabitants? Anthropogenic (human-caused) climate change is primarily caused by a large-scale warming of the planet. To understand how, this post reviews the basic science behind solar radiation coming into and out of the atmosphere—which drives our weather—and how heat flows between the atmosphere, land, water, and back into space. Then we will explore how greenhouse gases like carbon dioxide affect these heat flows and impact our climate, ending with how the built environment ties in.
In the interest of summarizing the science, this post does not contain the nuance, caveats, and details of the climate science and climate change found in more lengthy descriptions. The Intergovernmental Panel on Climate Change (IPCC)’s Fifth Assessment Report (AR5), along with its various appendices and references, provide much more detail and supporting research. An additional basic climate science overview can be found here.
The Greenhouse Effect: Trapping Solar Energy
Weather and climate (see here for the distinction) are fundamentally driven by the exchange of radiation between the earth, sun, and space. The Sun deposits energy throughout the day as solar radiation, while the earth eventually radiates that energy back into space; weather is the result of what happens to that energy in between. Energy is stored, transformed, and moved around, mostly in the form of heat.
The atmosphere is mostly transparent to incoming “short-wave” solar radiation (ultraviolet, visible, and near-infrared radiation): around 30% of the radiation coming from the sun is immediately reflected back out by clouds and the Earth’s surface. The remainder is absorbed by the atmosphere (1⁄3) and ground (2⁄3), turning to heat in the process.
After being absorbed, the energy is eventually released by the earth’s surface and atmosphere as “long-wave” radiation (mid- to far-infrared radiation), which the atmosphere is not transparent to. The atmosphere is “foggy” to most long-wave radiation, with the radiation being scattered around and/or absorbed; as much as 90% of the thermal radiation emitted at the surface simply ends up back at the surface. Absorbed radiation is eventually re-emitted, but the result of this absorption/re-emission process, along with the scattering, is that thermal energy only slowly diffuses through the atmosphere, trapping the energy within and keeping the atmosphere and surface warmer than it otherwise would be, in what is known as the greenhouse effect.
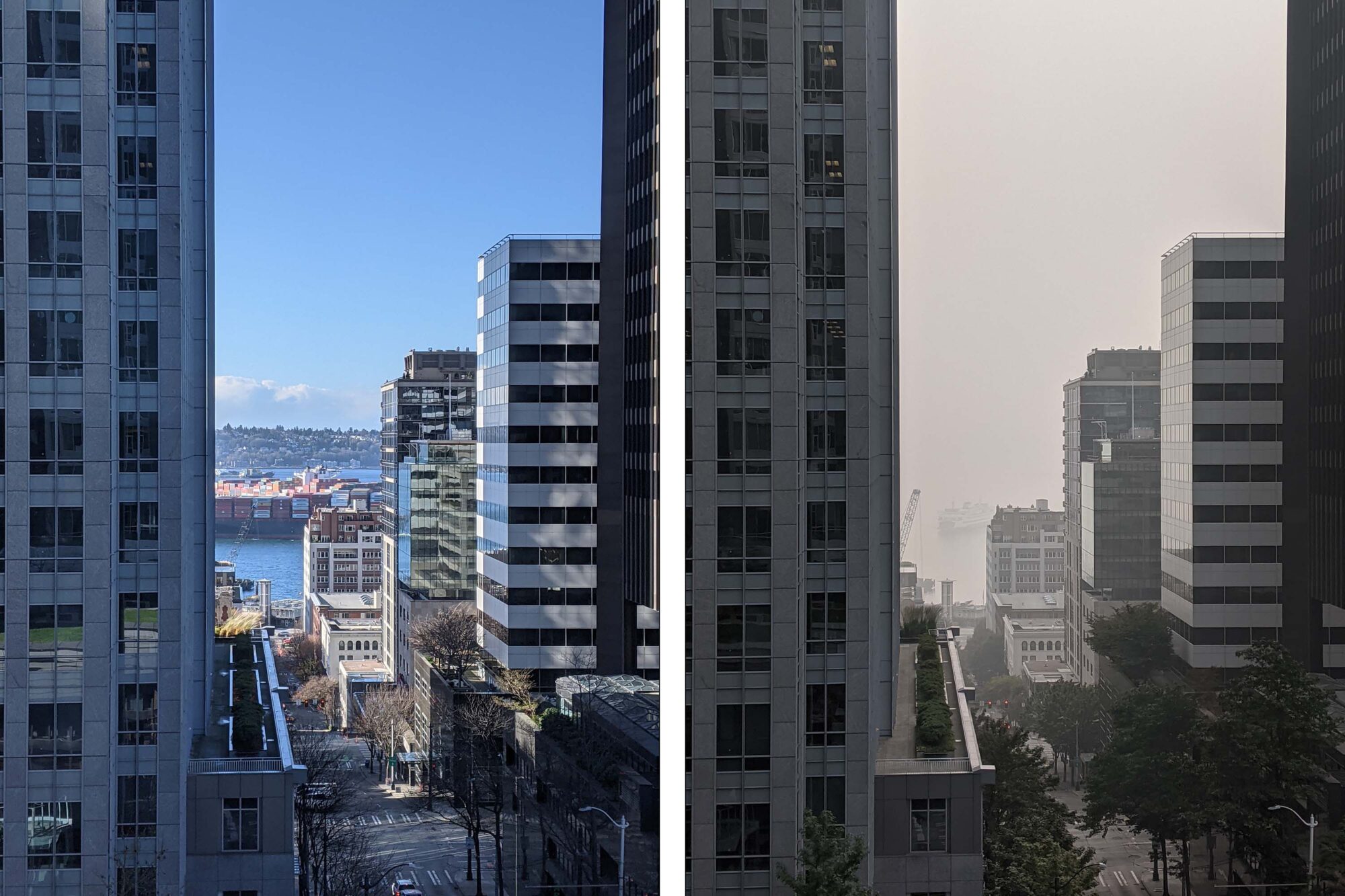
A clear versus foggy day in Seattle. The atmosphere is mostly transparent to incoming solar radiation, except for occasional clouds and fog near the surface. Although invisible to the naked eye, the atmosphere is always "foggy" for outgoing thermal radiation, which slows the escape of heat back into space. Human-caused greenhouse gases add to this fogginess and further slow the outward flow of heat. To thermal radiation, the atmosphere always looks like the right photo. Photo credit: Chris Savage / LMN Architects
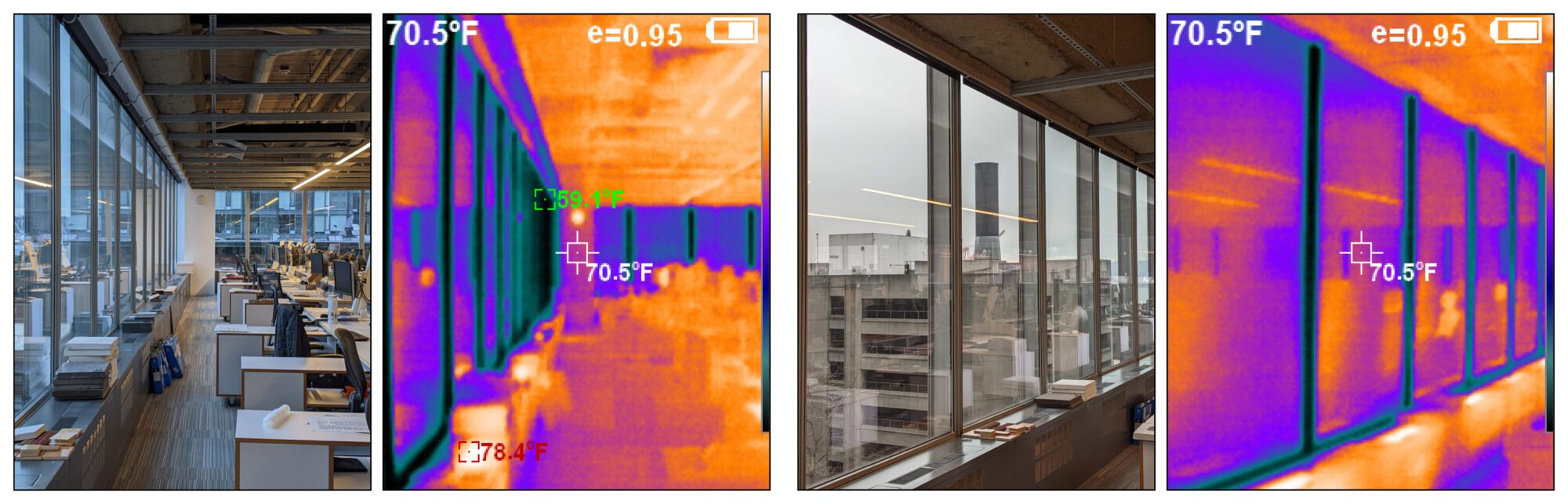
Photos capture short-wave radiation while thermal images capture long-wave radiation. As can be seen (or not seen) with the parking structure in the right panels, windows are transparent to visible light—a portion of the short-wave radiation band—while they are largely opaque to long wave radiation (although they can still be slightly reflective, as seen on the right: warm ceiling lights and an occupant can be seen in the reflection on the far right panel). This leads to the greenhouse effect that the AEC industry is more familiar with: solar energy easily enters through the glazing as short-wave radiation, but cannot escape once it turns to long-wave thermal radiation, with mechanical cooling systems often needed to remove the trapped heat.
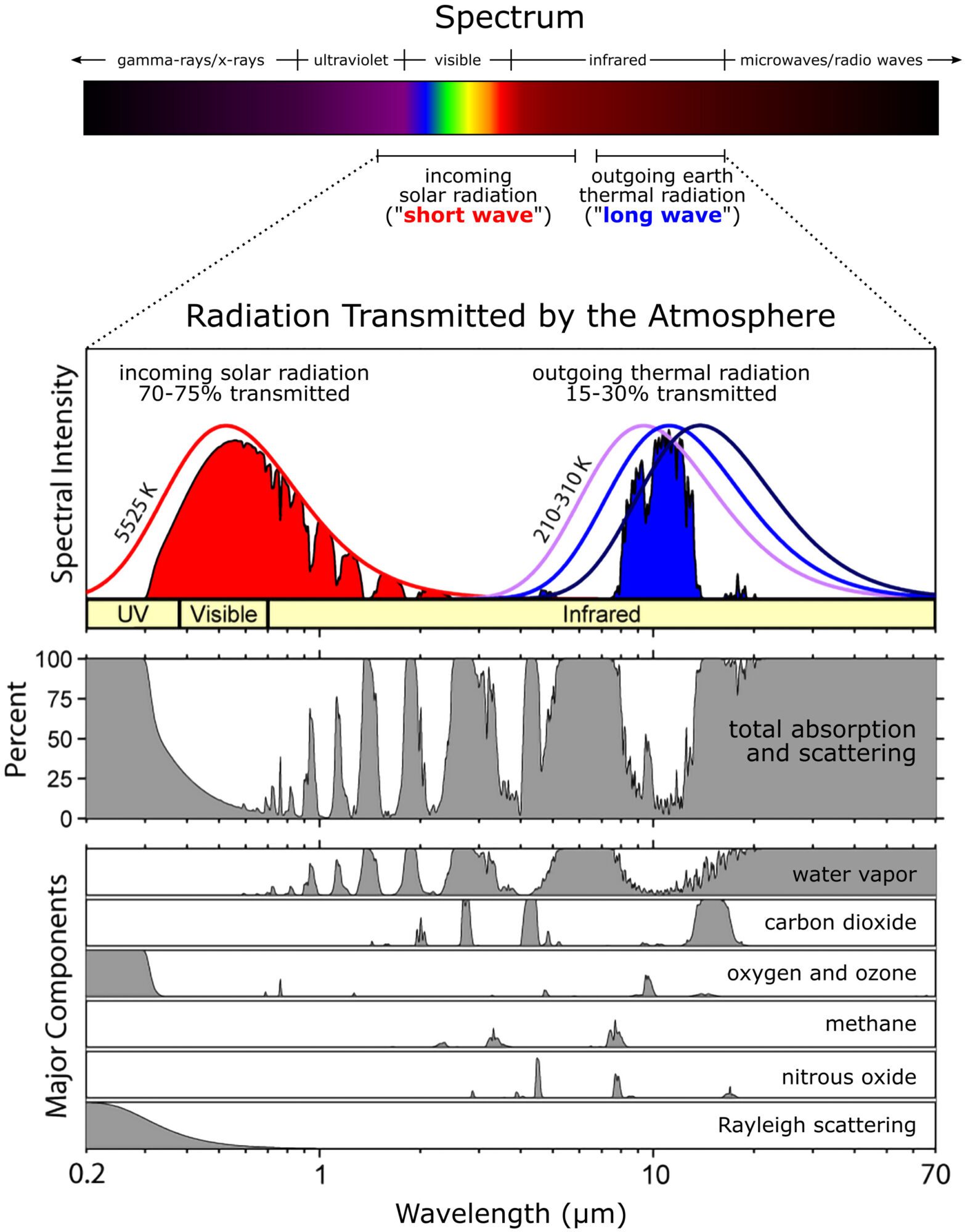
The radiation spectrum and transmission through the atmosphere. (top) Incoming solar radiation and outgoing thermal radiation are in different portions of the spectrum and behave differently in the atmosphere. The visible portion of the spectrum has been exaggerated. (bottom) Absorption and scattering in the short- and long-wave portions of the spectrum due to different gases in the atmosphere. Graphic credit: LMN Architects (spectrum/layout), Robert A Rohde (transmission panel) (Creative Commons Attribution-Share Alike 3.0 Unported)
Greenhouse Gases (GHG), Global Warming, and Climate Change
The nitrogen and oxygen molecules that make up the bulk (about 98%) of the atmosphere are transparent to long-wave radiation, but water, carbon dioxide (CO2), methane (CH4), and several other gases in the atmosphere are not. The latter components are referred to as “Greenhouse Gases (GHG)” as they are responsible for the heat-trapping effect in the atmosphere. Much like smoke from forest fires or smog from pollution can create a sky-obscuring haze even when only a very tiny part of the atmosphere (0.0001% or less by mass), the very small amounts of GHGs in the atmosphere are capable of significantly impeding the outgoing thermal radiation and creating the greenhouse effect. Water is the leading contributor to the greenhouse effect, but leaves a transparent opening in a portion of the long-wave spectrum that allows some thermal energy to escape the atmosphere. CO2 and other GHGs fill in some of this opening: the combination of water, CO2, and other GHGs create a far larger greenhouse effect than individual GHGs do.
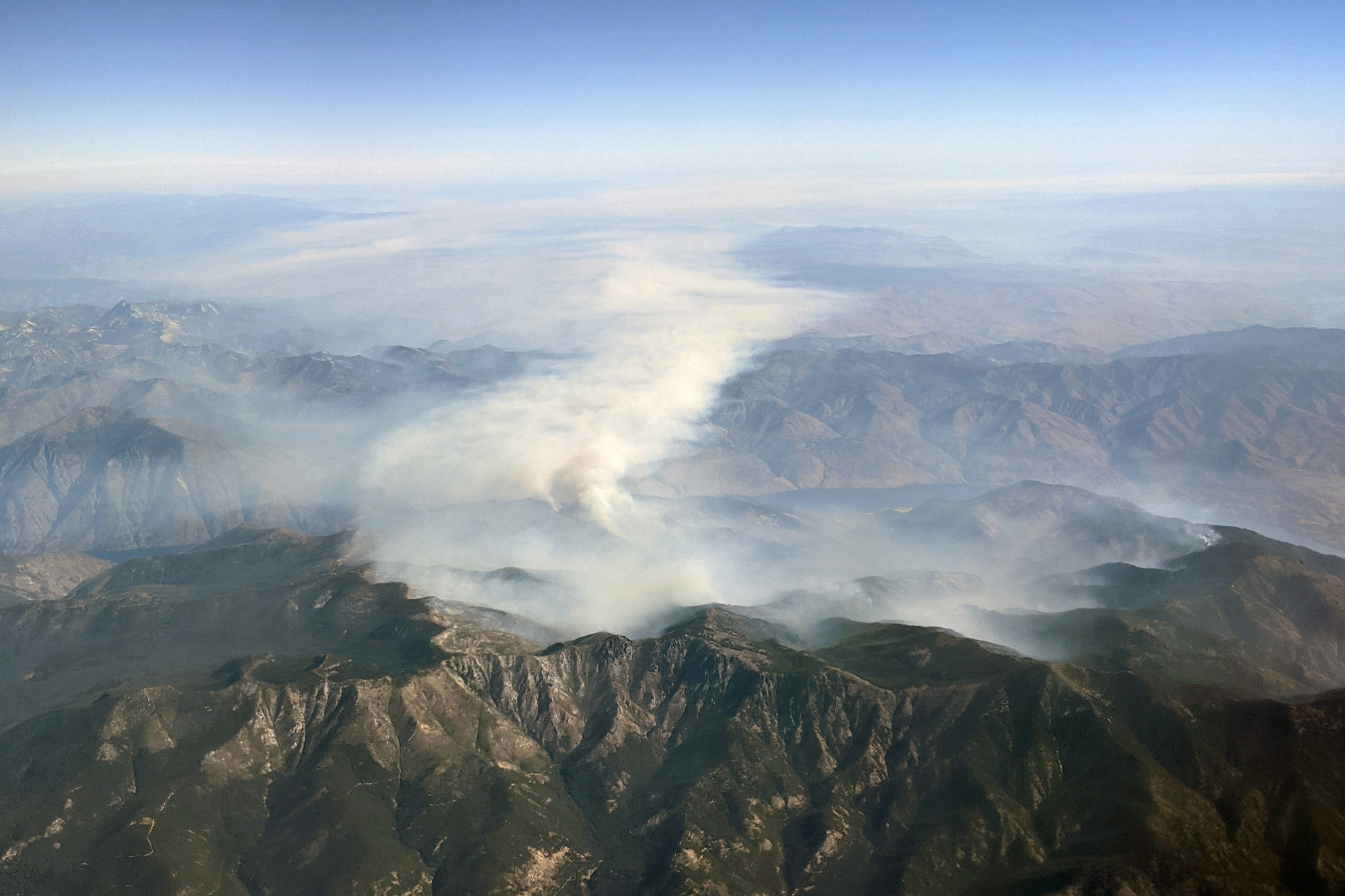
Smoke from forest fires (or smog from pollution) looks opaque even at very low levels; the smoke particles in the photo account for less than 0.0001% of the atmosphere by mass. Likewise, CO2 and other greenhouse gases can significantly inhibit long-wave radiation transmission through the atmosphere at very low levels, measured in parts-per-million (ppm). Photo credit: Chris Savage / LMN Architects
Humans have minimal direct effect on the atmospheric water levels: excess water added to the atmosphere by human activities such as steam generation is precipitated out within weeks (humans can indirectly affect atmospheric water levels through climate change, discussed later). CO2 levels, however, have been hugely affected by humans: combustion creates CO2 and humans have been burning things in large quantities for heat and power for a long time. CO2 does not break down in the atmosphere and is only slowly removed by biological processes on land and in the sea, with some also dissolving into water (which can store only finite amounts of CO2). These processes historically operated in equilibrium with naturally occurring CO2 emissions, e.g. CO2 emitted from the decay of dead plants and other biological material was extracted from the atmosphere by new plants and other biological growth. The rapidly increased use of fossil fuels over the last century has dumped a significant amount of CO2 into the atmosphere that the natural carbon cycle has been unable to absorb, leading to ever increasing CO2 levels and an increased greenhouse effect.
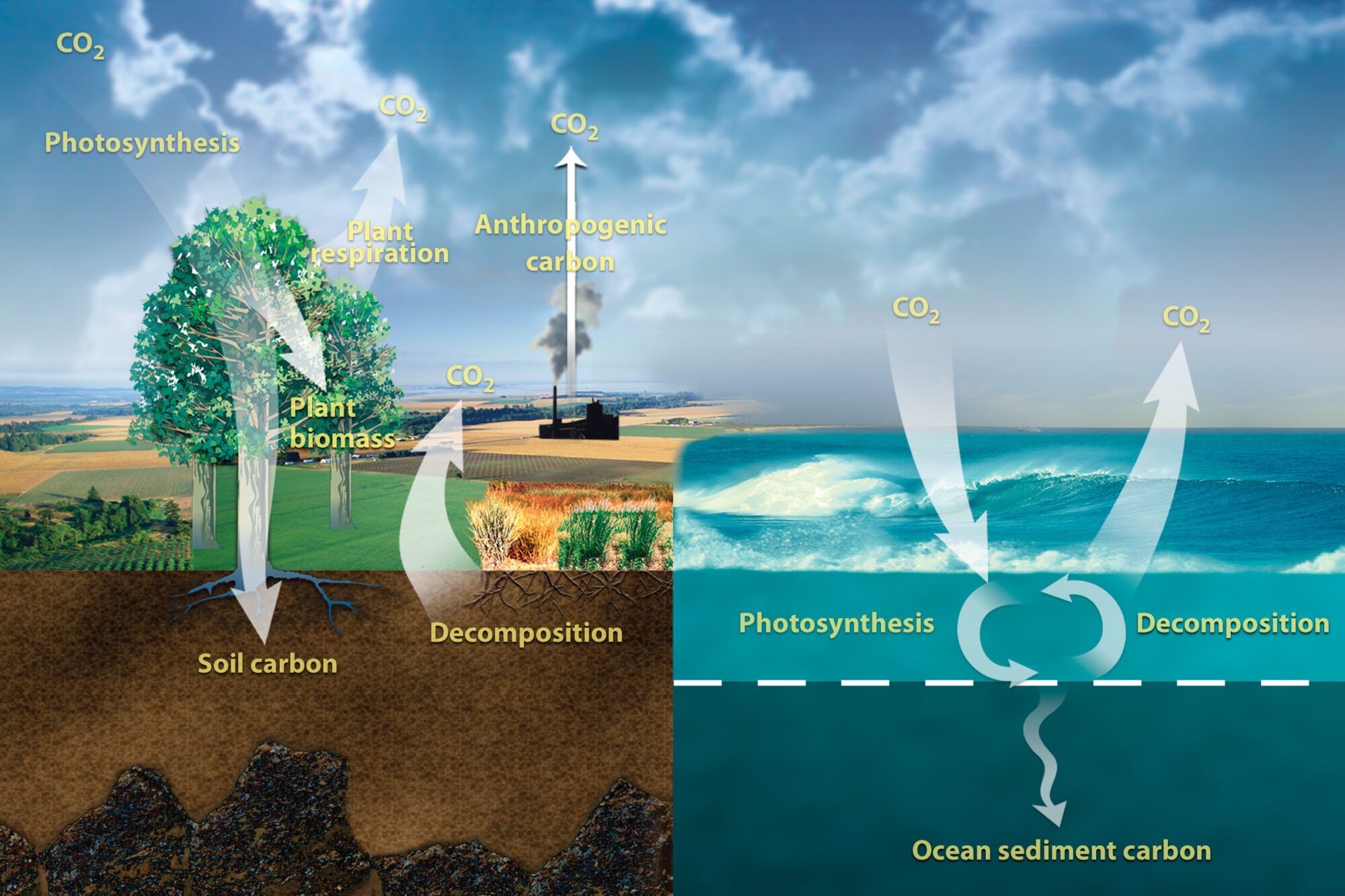
The carbon cycle: how carbon flows between the ground, plants, ocean, and atmosphere. Graphic credit: Office of Biological and Environmental Research of the U.S. Department of Energy Office of Science
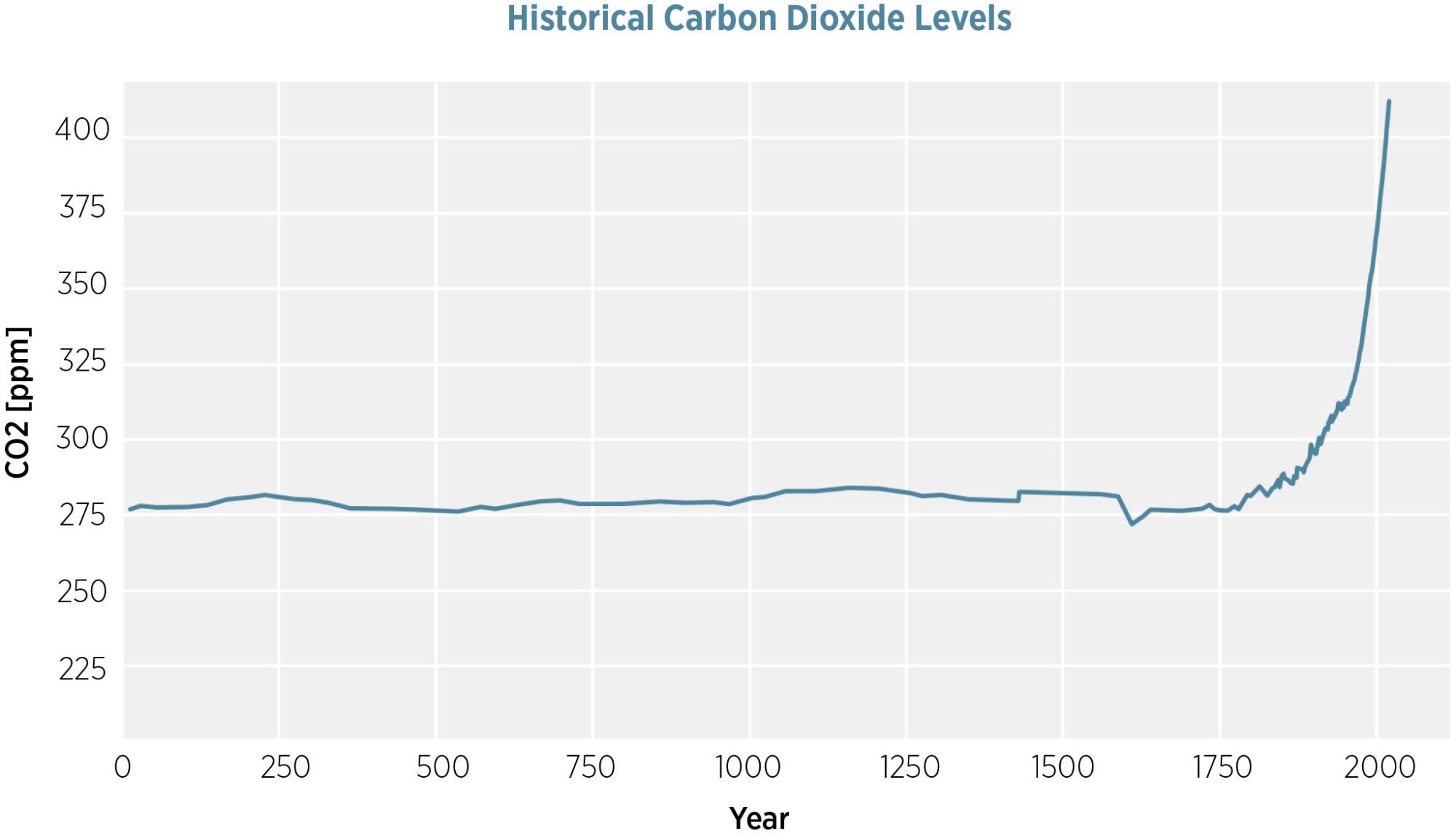
Historical CO2 concentrations, also known as the "hockey stick graph." Concentrations have risen noticeably during the industrial era, primarily due to the burning of fossil fuels, with a very rapid rise occurring during the last few decades. Data from Scripps Institute of Oceanography and MacFarling et al. (2006)
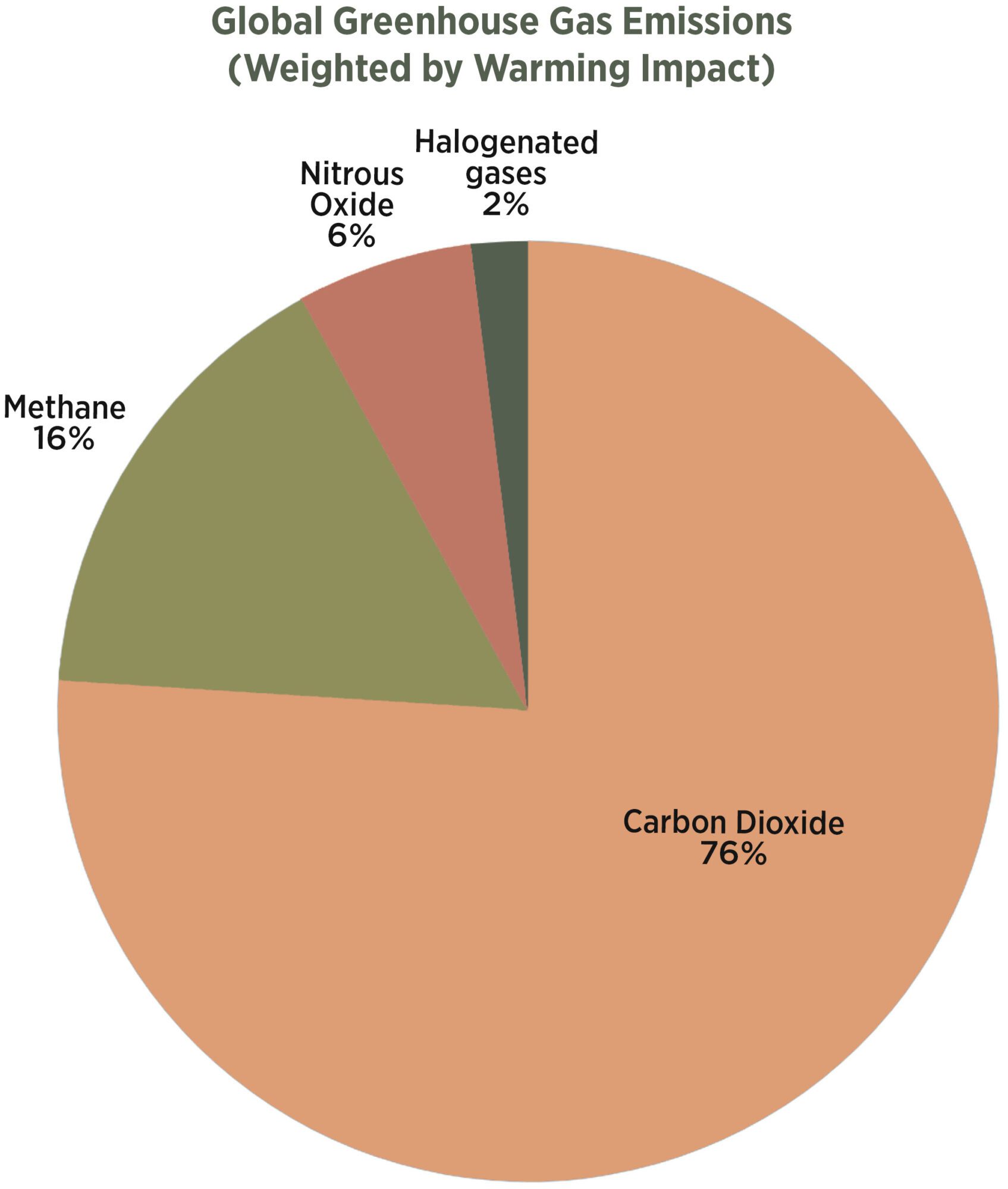
The overwhelming amount of CO2 from natural and anthropogenic (human caused) emissions means that the global warming contribution from CO2 dominates over that of other GHGs (other than water vapor). However, that does not mean other GHGs are not important: pound-for-pound, methane (CH4), nitrous oxide (N2O), and various refrigerants can be hundreds or thousands of times more effective at trapping heat in the atmosphere than CO2.
Apples-to-apples comparison of different GHGs are complicated by the fact that most of them break down or are otherwise removed from the atmosphere over time, some more quickly than others. A common means of making comparisons is the Global Warming Potential (GWP), which is the average warming impact (approximately) over a period of time, relative to the same mass of CO2 over that time; by definition, the GWP of CO2 is 1 for any timescale considered. A subscript is used to indicate the period, with some common periods being 20 years (GWP20) and 100 years (GWP100). GWP without a subscript is typically for a 100 year period.
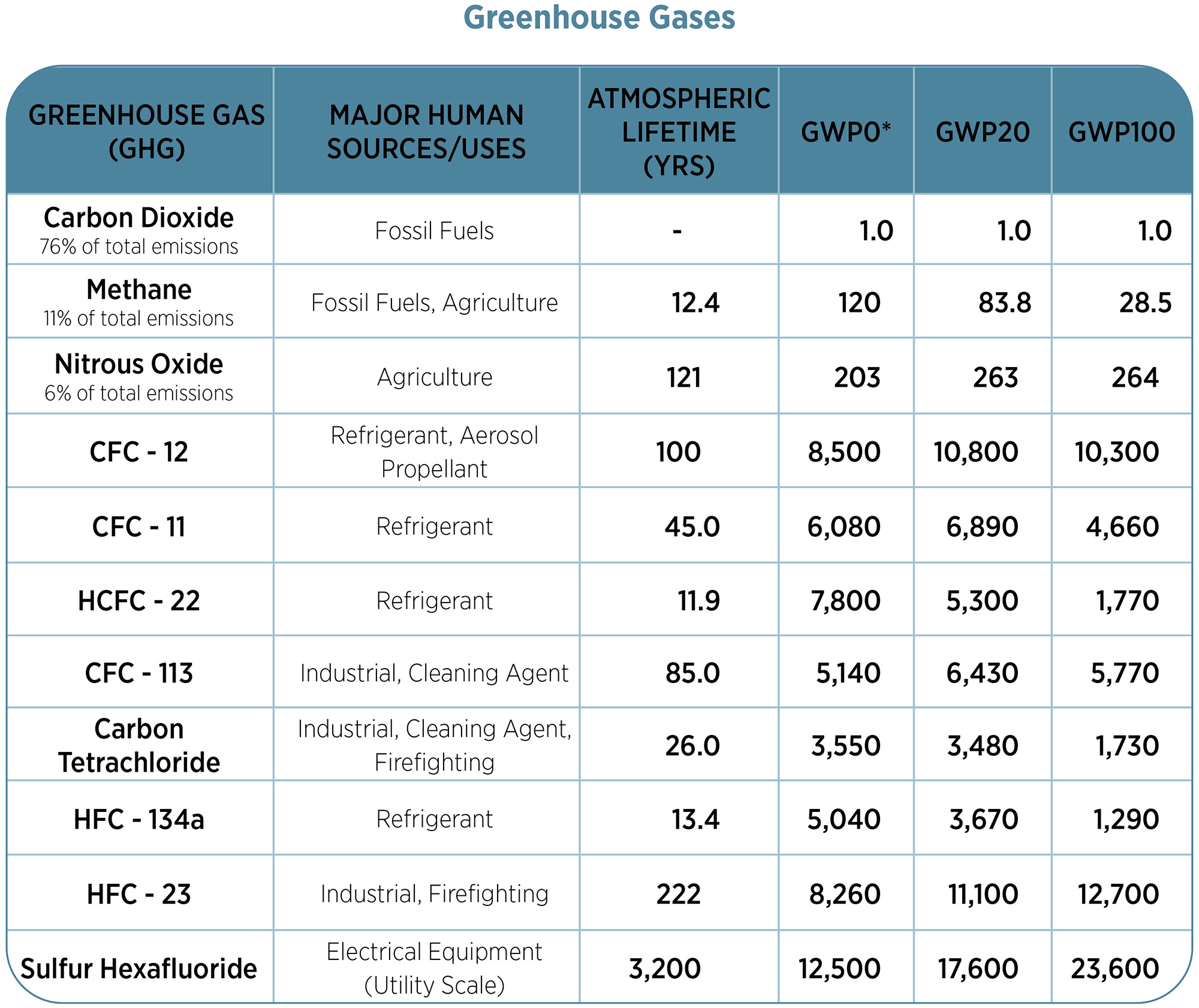
The global warming potential (GWP) of different greenhouse gases in the atmosphere, averaged over several time intervals. The GWP is relative to CO2. (*) GWP0 is the relative impact immediately after emission assuming the gas is well mixed in the atmosphere; in reality, it takes months or years for the gas to become fully dispersed. Data from the IPCC Fifth Assessment Report (AR5)
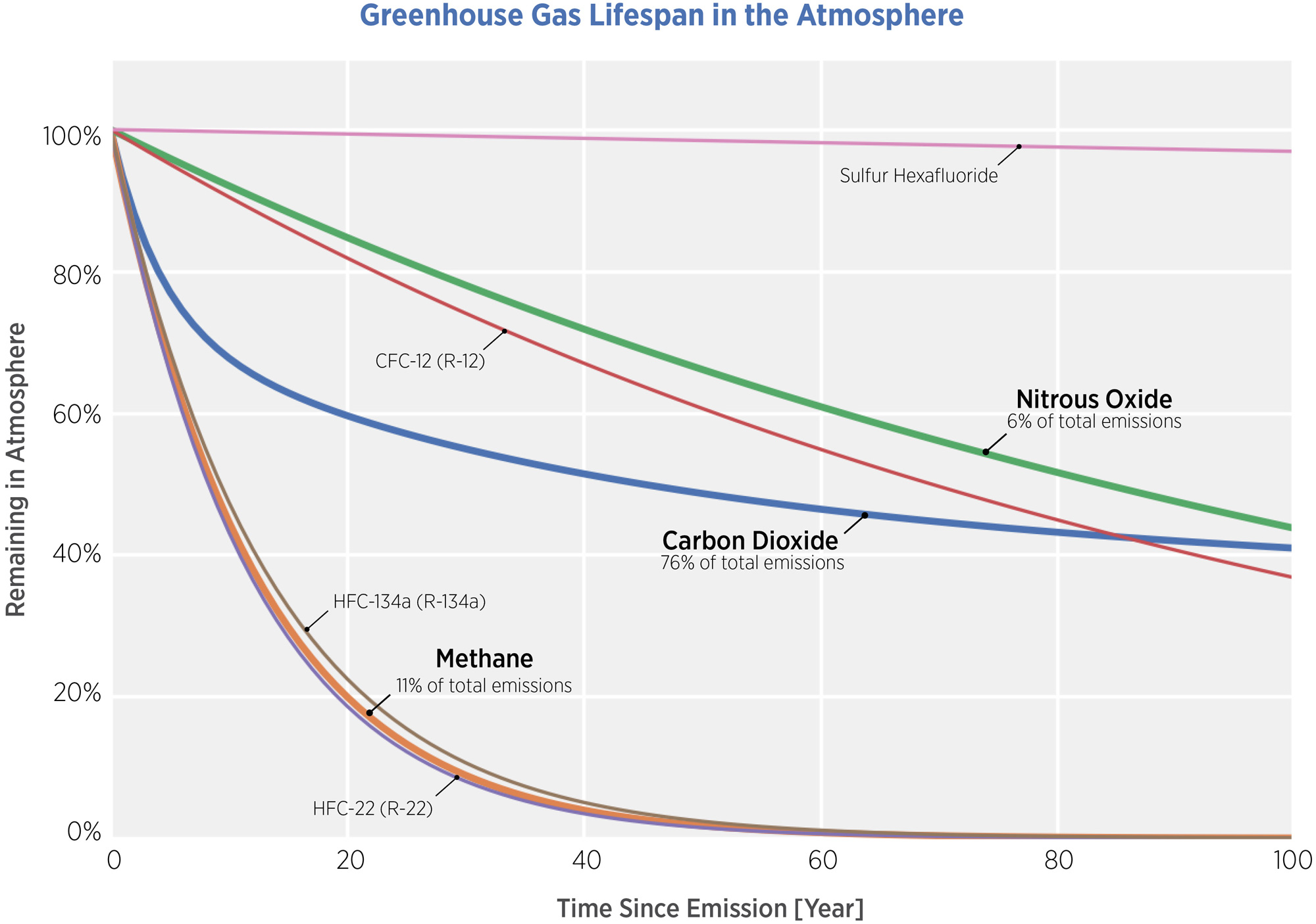
The amount of various greenhouse gases remaining in the atmosphere vs. time since they were released. Some gases, like methane, break down within a few decades, while other gases, notably some refrigerants, stick around for thousands of years. Data from the IPCC Fifth Assessment Report (AR5)
Weather, Climate and Heat Dynamics
The earth’s heat is a dynamic system and is never quite in thermal equilibrium: while the earth steadily loses heat to space, the quantity of incoming solar radiation changes throughout each day, also varying by season as the earth orbits our sun on a 23° axial tilt. In addition, heat is stored and released by the ground and bodies of water (referred to as thermal reservoirs). The exchange of heat between these components is the main underlying driver of short-term weather and longer-term climate patterns. Weather adds a layer of complexity to this basic story of heat dynamics in a couple ways. Clouds, snow, and ice reflect more of the incoming solar radiation due to their high albedo, reducing the amount of energy being absorbed by the earth. Large scale wind patterns move heat and moisture between locations. Radiation ultimately drives the weather patterns, and weather, in turn, affects the radiation and thermal balances. While each component of this story has a firm scientific understanding, their complex interactions mean that detailed scientific modeling is required to make short-term weather forecasts and longer-term climate predictions. In the remainder of this section, we look in more detail at one part of the story: how heat flows around between the atmosphere, ground, and oceans.
The atmosphere can shed excess heat fairly quickly, in only hours or days, which is why temperatures can drop rapidly once the sun goes down. By itself, the atmosphere also responds to increased levels in GHGs over the same time scale: it takes only days for average temperatures to approach new, elevated levels corresponding to the amount of GHGs in the atmosphere. However, the atmosphere is thermally coupled to the ground and bodies of water, which take much longer to heat or cool—on timescales of months or years—due partly to the very large amounts of energy it takes to change their temperature compared to the atmosphere (they are thermal reservoirs). Other than a very thin layer of the surface (such as hot asphalt in the summer), these non-atmospheric thermal reservoirs vary in temperature very little throughout the day, typically less than a degree.
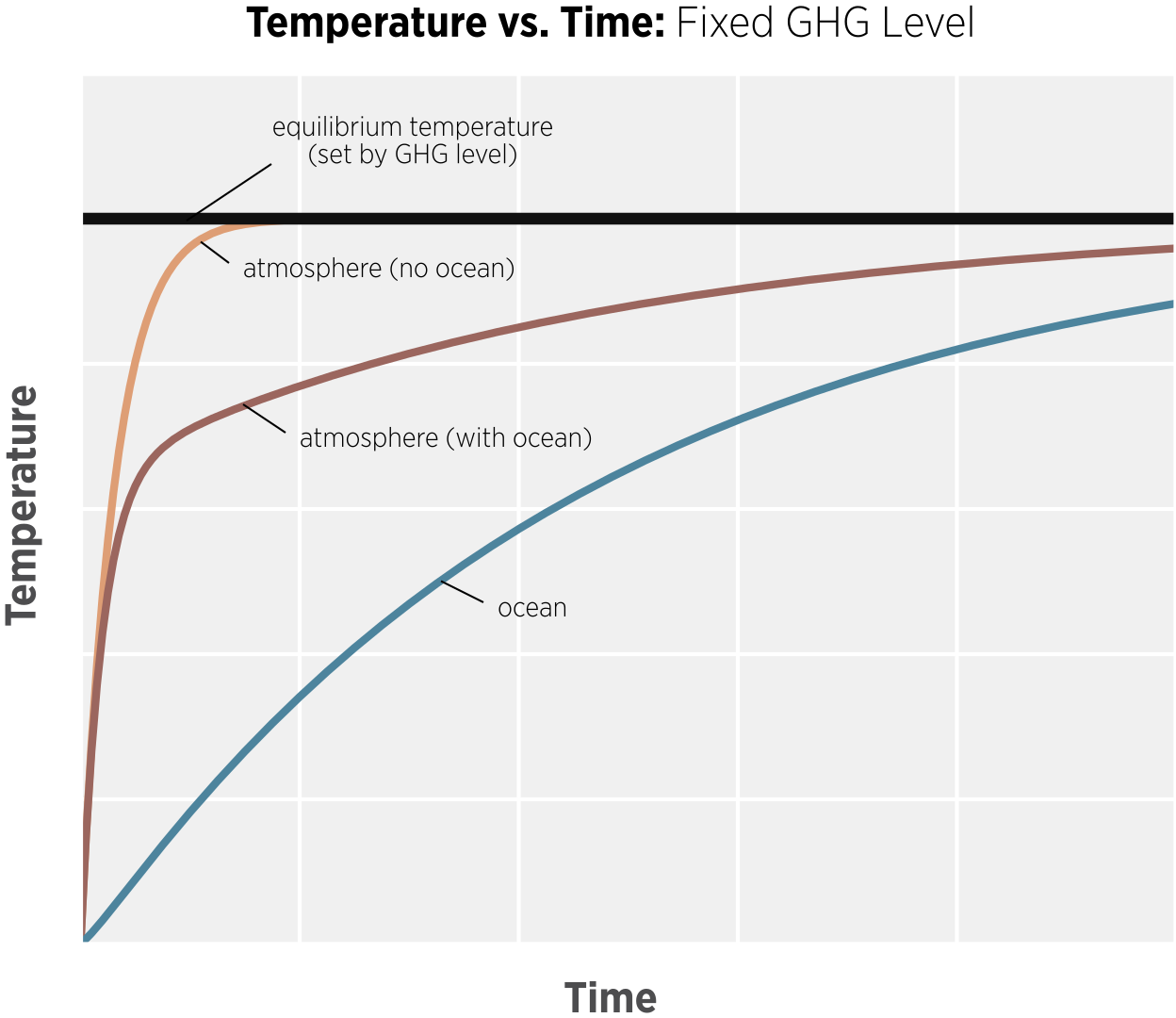
How quickly it takes the atmosphere to reach temperature equilibrium from increased GHG levels, with and without the large thermal mass of the oceans. The equilibrium temperature is the temperature at which the atmosphere and/or ocean are in thermal balance: the incoming solar energy is exactly counterbalanced by the outgoing thermal radiation. Heat dynamics drive a body’s temperature to the equilibrium temperature but, because thermal masses must store or release large amounts of energy to change temperature, it takes time to reach equilibrium: for the atmosphere (small thermal mass) it takes only days, while for the oceans (large thermal mass), it can take decades.
These much more slowly evolving thermal reservoirs tend to mitigate the daily and seasonal atmospheric temperature swings. They also play a large role in when our seasons occur: without these reservoirs, the hottest day of the year in the northern hemisphere would always be around the summer solstice (around June 21 in the northern hemisphere), the day which receives the greatest amount of solar radiation. Instead, water bodies and land slowly heat up during the spring and summer, often reaching peak temperatures months after the solstice.
One of the most important heat reservoirs is the oceans, which can store huge amounts of heat and can take decades or longer for their temperatures to come to equilibrium with changing conditions. The oceans have their own heat dynamics, such as the El Nino–Southern Oscillation, and are thermally coupled directly or indirectly to nearly every region of the Earth: thermal changes in the oceans have wide-ranging impacts on the weather/climate throughout the globe and can continue to do so for long periods of time.
Climate Change and Feedback
Global warming leads to climate changes other than a simple warming of the temperature. Because weather patterns are tied to the radiation balance and heat reservoirs, global warming can alter cloud and rain patterns, which impacts farm production and water availability, among other things. Changes in the ocean temperature can affect ocean currents that move heat around: despite being at latitudes more comparable to Canada, the western European climate is closer to that of the United States due to the Atlantic currents bringing tropical heat up from the south; a shutdown of this current (which has occurred in the past) can conversely lead to a significant localized cooling of western Europe. Warming leads to more extreme weather events such as heat waves, cold waves, hurricanes, etc. Recent occurrences of “polar vortex” cold snaps in the U.S. and Canada may be related to loss of Arctic sea ice, which can weaken air circulation patterns that tend to restrict the cold Arctic air to the polar regions; the Arctic becomes warmer while the mid-latitudes become cooler when those air circulation patterns break down.
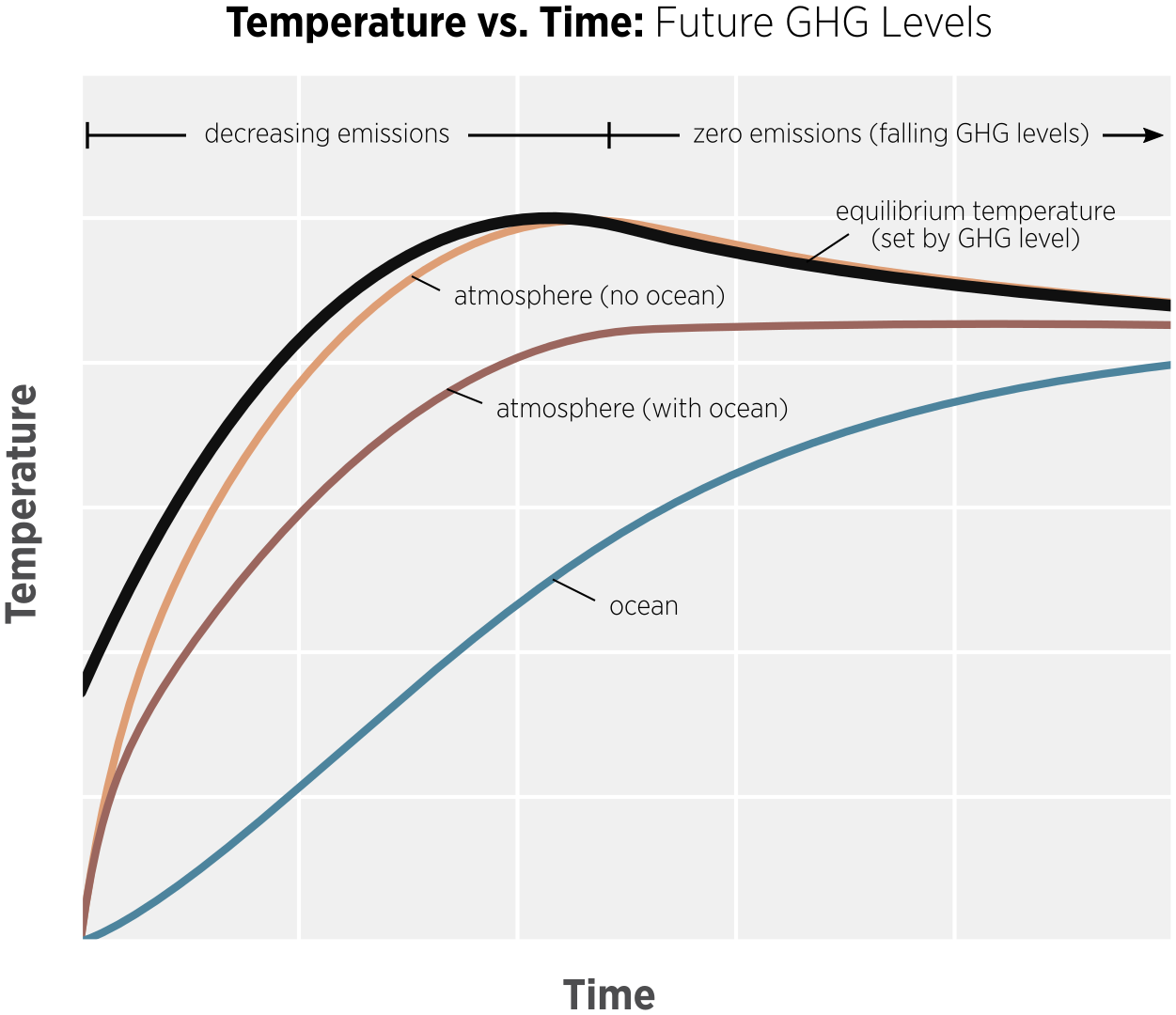
The atmospheric temperature with and without the effect of the oceans as GHG emissions are reduced to zero. After emissions are halted and GHG levels begin to fall, the atmosphere’s temperature can remain relatively flat as the oceans continue to warm, counter-acting the falling greenhouse effect. Significant cooling of the atmosphere only starts to occur after the oceans have finished warming.
Some of these climate changes can further exacerbate the warming problem. Higher temperatures lead to melting of high albedo glaciers, ice, and snowpacks, which means even more solar radiation will be absorbed. Higher temperatures allow for more water vapor in the atmosphere, increasing the greenhouse effect. Thawing of the permafrost releases embedded methane, also increasing the greenhouse effect. These feedback effects ultimately lead to more global warming than expected directly from the extra anthropogenic-emissions-induced atmospheric heat trapping.
The ocean has been slowly warming over the last centuries, but due to its huge thermal mass, it has not kept up with the warming in the atmosphere. Even if GHG emissions were halted and the atmospheric warming stopped, the ocean will continue to warm for decades or centuries until it comes into equilibrium with the elevated atmospheric temperatures, warming the earth by as much as another degree Fahrenheit. If we halt our emissions, the cooling due to falling GHG levels in our atmosphere would approximately cancel the warming effect due to the ocean for many decades, but it would be some time before global warming begins to reverse.
Impact of the Built Environment
From Post 01, we saw that buildings cause around 40% of human GHG emissions, from carbon emitted to produce the materials used to construct them, to fossil fuels burned to heat them and provide electricity. While these GHG sources are often the primary focus for designers looking to reduce their carbon impact, designers can still influence related GHG emissions that are not typically attributed to the buildings they design. For example, designers can reduce vehicle-related emissions—from supplying buildings and getting occupants to and from there—by locating buildings closer to mass transit and providing electric vehicle (EV) charging infrastructure to expand the use of EVs in support of eliminating the internal combustion engine. Civil infrastructure used to serve these buildings—such as roads, sewer lines, and power plants—also have a GHG footprint and reducing the need for this infrastructure will reduce their corresponding carbon impacts. Designers wishing to have the most impact on climate change should consider these and other emissions that stem from beyond the project footprint and not solely emissions that are customarily considered to “belong” to their building.
Most of the associated emissions are carbon dioxide from combustion, but methane leaks from appliances, gas pipelines and gas extraction are also important; turning to renewable energy for both power and heating will reduce or eliminate many of these emissions at the site and farther up the construction supply chain. Due to their high GWP, halogenated refrigerants used in mechanical equipment and off-gassing from construction materials can be significant.; if lower-GWP replacements are not developed and used, these halogenated compounds will account for a larger and larger fraction of the building GHG footprint. Even now, leaking refrigerants can have a greater warming impact than the energy-related emissions in supermarkets and facilities with extensive refrigeration equipment. While carbon dioxide remains the primary target for emission reduction, other GHGs should not be ignored.
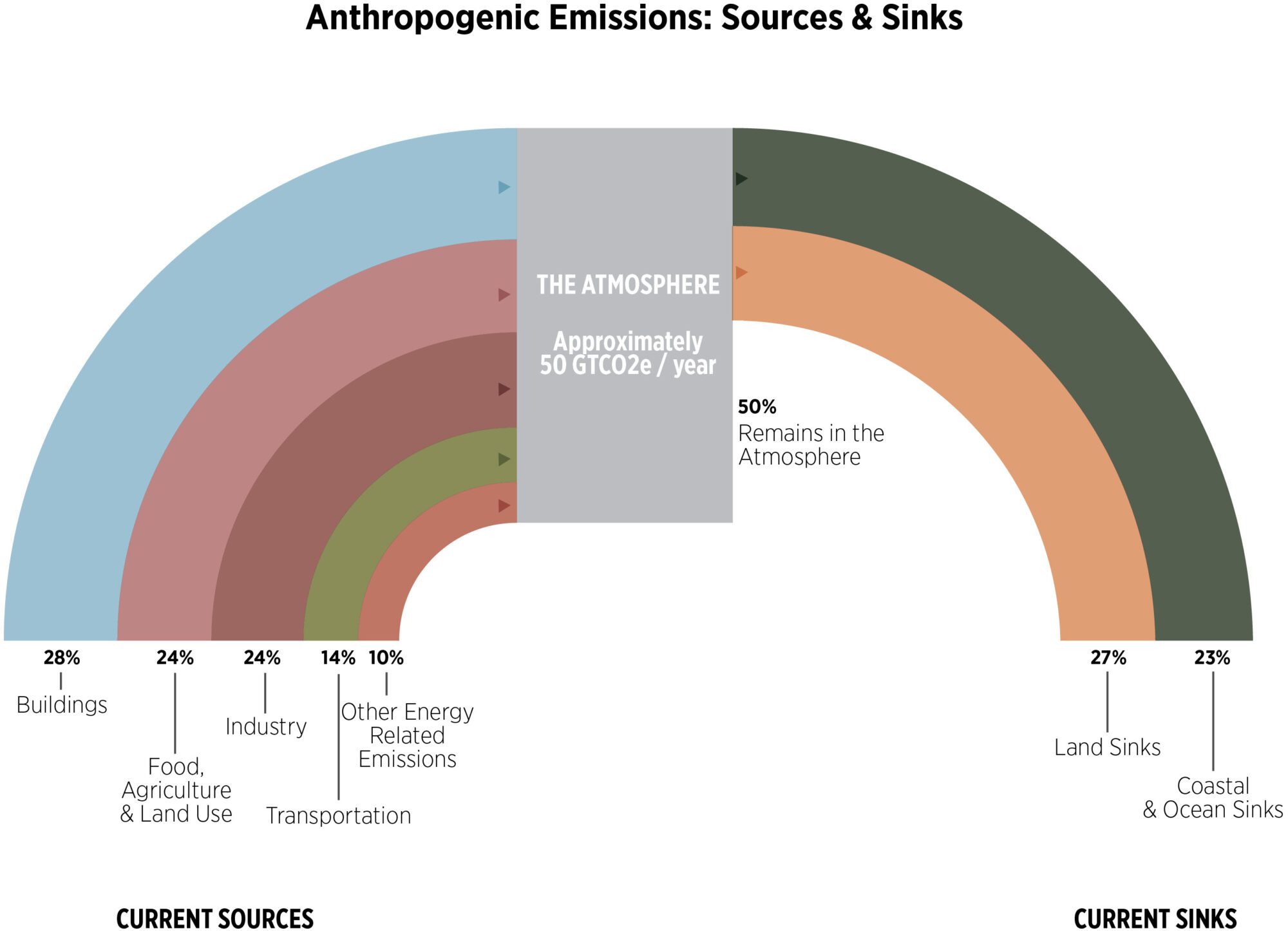
Where human-induced greenhouse gas emissions come from and where they go. About half of the emissions will remain in the atmosphere, causing levels to increase over time. Based on this Project Drawdown graphic, updated with data from Architecture 2030.
Beyond the global warming induced by GHG emissions, the built environment can lead to localized warming in other ways. Urban heat islands, where urban areas can have ambient temperatures 1 to 7 °F warmer than surrounding areas, is an example. The large amount of asphalt and other dark materials that absorb solar radiation rather than reflect it leads to additional heat being accumulated in urban areas; higher-albedo roadways and rooftops can alleviate the issue. The relative lack of trees also has an impact as trees, in addition to providing shade, can mitigate daily temperature swings through evapotranspiration, a process in which some of the solar energy goes into evaporating water instead of heating the air. Combined with global warming, these other effects can lead to large, localized warming in the urban areas where most humans reside, even reaching unsafe or intolerable levels of heat that neither global nor local warming would achieve on their own.
Global warming is complex. The summary is meant to provide a baseline of knowledge to understand the physical systems that regulate global temperatures, as well as key terminology and concepts that will be used throughout the series. The next post covers risk and the time value of carbon.
Please email any questions or comments to Kjell Anderson, kanderson@lmnarchitects.com
Thanks to our external collaborators and peer reviewers
Chris Hellstern, Miller Hull; Dan Jaffe, UW; Mae Gustin, University of Nevada-Reno; Seonhee Kim, Design Collective; Ryan Davies, Macalester College
LMN Architects Team
Huma Timurbanga, Justin Schwartzhoff, Jenn Chen, Chris Savage, Andrew Gustin, Kjell Anderson
Posted: 10/10/2022
The text, images and graphics published here should be credited to LMN Architects unless stated otherwise. Permission to distribute, remix, adapt, and build upon the material in any medium or format for noncommercial purposes is granted as long as attribution is given to LMN Architects.